- 1Department of Urology, Affiliated Hospital of Guizhou Medical University, Guiyang, China
- 2Department of Urology and Institute of Urology, West China Hospital, Sichuan University, Chengdu, China
- 3Department of Otorhinolaryngology, The Ninth People’s Hospital of Chongqing, Chongqing, China
- 4Department of Urology, Guizhou Provincial People’s Hospital, Guiyang, China
A circadian rhythm is an internalized timing system that synchronizes the cellular, behavioral, and physiological processes of organisms to the Earth’s rotation. Because all physiological activities occur at a specific time, circadian rhythm disturbances can lead to various pathological disorders and diseases. Growing evidence has shown that the circadian clock is tightly connected to male fertility, and circadian perturbations contribute to infertility. The night shiftwork, insufficient sleep, and poor sleep quality are common causes of circadian disturbances, and many studies have reported that they impair sperm quality and increase the risk of male infertility. However, research on the impacts of light, body temperature, and circadian/circannual rhythms is relatively lacking, although some correlations have been demonstrated. Moreover, as the index of sperm quality was diverse and study designs were non-uniform, the conclusions were temporarily inconsistent and underlying mechanisms remain unclear. A better understanding of whether and how circadian disturbances regulate male fertility will be meaningful, as more scientific work schedules and rational lifestyles might help improve infertility.
1 Background
As the most predictable environmental change in our planet, day/night alteration is accompanied by oscillations in environmental temperature, humidity, and food availability (1). When the sun rises in the morning, diurnal animals awaken from sleep and plants are exposed to the first light of a new day; thus, all organisms have evolved a universally internal rhythmic timing system to adapt to the Earth’s rotation (2–4). This endogenous system is called circadian rhythm, which is a precise 24-h oscillating cycle to guarantee optimal performance for biochemical functions and physiological processes (1, 5), including sleeping habits, body temperature, feeding behavior, hormone secretion, diet (6, 7) and homeostasis (7–9). A recent paper published in Nature also showed that time-restricted feeding (TRF) delayed aging and extended lifespan through circadian-regulated autophagy (10).
Before artificial light was invented, humans modified their behavior to match the natural alteration of day/night (3). Although advancements in modern science and technology have greatly changed our daily living habits and improved human health, they are also accompanied by increased diseases (11). Nowadays, elevated social pressures, overloaded work schedules, and personal habits have caused individuals extensively living with artificial lights or luminescent screens (11) that one-fifth terrain in the planet and four-fifth of the world population (99% individuals for Europe and USA) are exposed to light-populated skies or receive misdirected and obtrusive artificial/outdoor light (12). Meanwhile, prolonged night shiftwork, circadian sleep disorders, jet lag, and distance travel across multiple time zones have become more common (11). All these habits inevitably change the daily wake/sleep cycle and gradually lead to circadian misalignment (11), which finally disturb homeostasis, induce oxidative stress, promote an inflammatory response, and accelerate the coagulation process (7–9, 13). These populations are also more vulnerable to hypertension, diabetes mellitus (DM), hyperlipidemia, obesity, atherosclerosis, and other diseases (7, 11, 14, 15).
Fertility is a fundamental process in animal reproduction and is affected by environmental cues such as light, temperature, rainfall, and food availability (5, 16–19); as such, most species reproduce in times with mild weather and optimal food availability (5, 20). For example, many animals evolve a precise seasonal fertility between spring and early summer; others with longer gestations mate in late summer and autumn, which leads to pregnancy in winter and parturition in spring (21–23). Although humans are not seasonal breeders, their sexual activity (24, 25) and reproductive function are still influenced by the peripheral environment (24, 26), although relatively less (27, 28). Infertility is defined as the inability to successfully spontaneously conceive for at least 2 years of unprotected sexual intercourse and is influenced by occupational and lifestyle factors such as smoking, obesity, alcohol consumption, psychological stress, and lack of exercise (29, 30). Currently, infertility affects nearly 15–20% of all couples worldwide (24, 30) and contributes to various psychological, medical, and financial consequences (30).
In fact, numerous studies have shown that elevated night shiftwork, overloaded work schedule, poor sleep quality, and popularization of mobile phones disrupted the circadian rhythm (5, 27, 28, 31, 32) to impair female fertility by affecting the menstrual cycle and altering hormone secretion, which finally increases the incidence of preterm birth, spontaneous abortion, and membrane rupture and reduces breastfeeding success (1, 23). However, males are estimated to be solely responsible for 20–30% cases of infertility (30, 33), while other studies have even indicated a rate of 50% (34). Nevertheless, whether disturbed circadian rhythms contribute to male infertility, like deteriorating female reproduction, is controversial, and whether a normal or healthy circadian rhythm improves male fertility is more interesting. Herein, we review and discuss the potential relationship between the circadian rhythm disturbances and male fertility.
2 Organization and molecular mechanism of the circadian clock system
Circadian rhythm was first described by Konopka in 1971, who cloned the mutant clock gene of Period in Drosophila; the veil of the circadian clock was subsequently disclosed (1, 35). Recently, the 2017 Nobel Prize in Physiology or Medicine was awarded to Jeffrey C. Hall, Michael W. Young, and Michael Rosbash to reward their huge achievements in exploring and clarifying the regulatory mechanisms in the circadian clock.
The circadian clock system consists of three basic elements: the input pathway, the main oscillator, and the output pathway. The input pathway is a passage that modulates the non-24-h central circadian pacemaker to 24-h and adapts the endogenous timing phase to local environment (1). As the main Zeitgeber that synchronizes organisms’ circadian clock (9, 36, 37), environmental light is perceived by melanopsin-containing retinal ganglion cells and is transmitted to a central oscillator via the retinohypothalamic tract as electrical signal (38) (Figure 1). Other factors, such as environmental temperature, feeding behavior, physical exercise, and social interactions, can also be perceived (38). The master oscillator of the circadian clock for mammals, which is composed of a set of circadian clock genes and coded proteins, is located in the suprachiasmatic nucleus (SCN) of the anterior hypothalamus which acts by manipulating the daily endogenous rhythms of physiological and behavioral processes (9). The human SCN comprises approximately 50,000 neurons, including diverse cellular components and numerous neurotransmitters and peptides (39, 40). In addition to the main oscillator, the circadian clock system also exists in almost every peripheral tissue and organ (1, 3, 41). For example, when feeding behavior occurs beyond regular eating time, the circadian timing system in SCN is constant, but rhythms in peripheral organs such as stomach, liver, and pancreas are altered and disturbed, which finally contributes to desynchronization between the internal and peripheral circadian clocks (38). Finally, the output pathway transfers central circadian information to peripheral effector organs (1). The output neural pathways are diverse and include the paraventricular nucleus of the thalamus, subparaventricular zone, medial preoptic area, and dorsomedial nucleus of the hypothalamus (1, 42–46). Hormones are also regulated by the SCN rhythm and are regularly secreted during the day/night cycle (1, 47, 48).
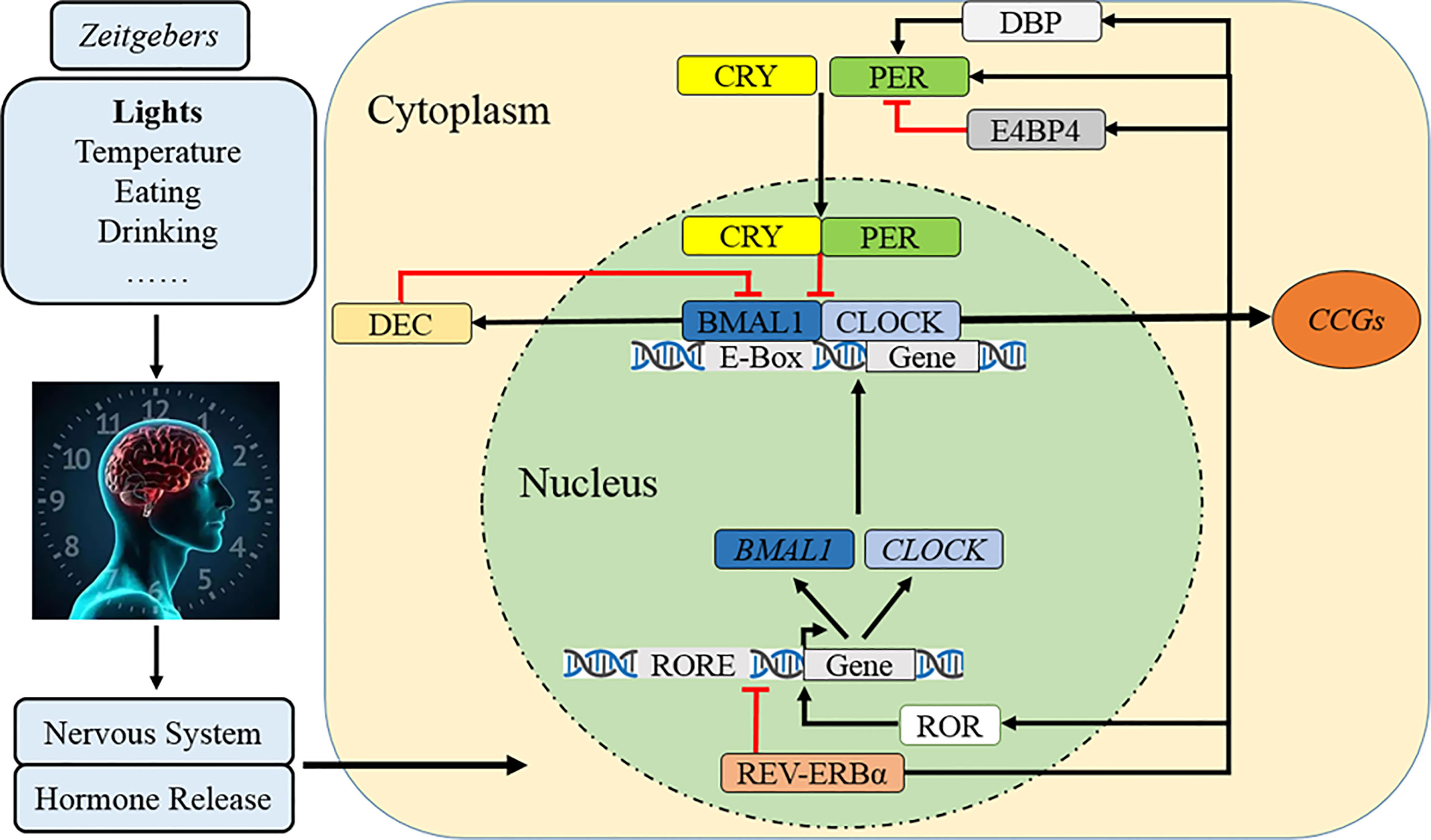
Figure 1 Molecular mechanism of the circadian clock. After cues from Zeitgebers are transmitted to SCN as electrical signals, the circadian clock rhythm is orchestrated by a set of genes and proteins which forms interlocked positive and negative feedback loops. Specifically, CLOCK and BMAL1 constitute the core positive heterodimer complex (CLOCK/BMAL1) to activity rhythmic transcription of PER1/2/3, CRY1/2, and relevant clock controlled genes (CCGs). The repressors of PER and CRY accumulate and form a negative feedback loop in cytoplasm during the day and then translocate back to the nucleus to inhibit the level and activity of CLOCK/BMAL1. The complex of PER/CRY is also subsequently disassembled or resolved, while a new circadian cycle is also followed by another 24–h. Meanwhile, REV-ERBα reduces BAML1 transcription and RORα promotes it to manipulate the CLOCK/BMAL1 complex. The third loop involves that DBP induces PER transcription and lengthens the rhythmic period but E4BP4 exerts opposite effects, while DEC inhibits CLOCK/BMAL1-induced PER1 transcription.
At the molecular level, the circadian clock system is orchestrated by a set of genes and proteins that form interlocking positive and negative feedback loops of transcription and translation (11, 49) (Figure 1). In the nucleus, the circadian locomotor output cycle kaput (CLOCK) and brain and muscle ARNT-like protein 1 (BMAL1) constitute the core positive transcription factor (CLOCK/BMAL1) (1, 3, 11, 49). This heterodimer complex binds to a specific E-box (5′-CACGTG-3′) sequence on the promoters of Period (PER1, PER2, and PER3), Cryptochrome (CRY1 and CRY2), and relevant clock-controlled genes (CCGs), which finally activate their rhythmic transcription (1, 3, 11, 49). The PER and CRY accumulate and dimerize in the cytoplasm during the day and then translocate back to the nucleus with a timed delay to inhibit the activity of CLOCK/BMAL1 and downregulate the transcriptional activation of downstream circadian genes, forming the core negative feedback loop (1, 3, 7). The repressor complex of PER/CRY is also subsequently disassembled or resolved, while a new circadian cycle occurs in the subsequent 24–h (1, 3, 7). The secondary negative regulatory arm involves the nuclear receptors of orphan nuclear receptor (REV-ERBα/β) reducing BAML1 transcription and retinoic acid-related orphan receptor alpha (RORα/β) promoting it (7, 50, 51). Another CLOCK/BMAL1 negative loop comprises D-site albumin promoter-binding protein (DBP) and E4 promoter-binding protein 4 (E4BP4) which compete binding to the PER promoter of the D-box element (52–54); the upregulated DBP cooperatively induces PER transcription and lengthens the rhythmic period but E4BP4 exerts opposite effects (52, 54), while elevated differentiated embryo chondrocyte (DEC) inhibits CLOCK/BMAL1-induced PER1 transcription by direct protein–protein interactions with BMAL1 and/or competition for E-box elements (55, 56).
3 Light exposure and male fertility
Light is a fundamental Zeitgeber that synchronizes endogenous circadian rhythms with external environments (57). Nowadays, millions of televisions, computers, and smartphones are operated every day (17), and the time spent on these devices peaks in the evening and shortly prior to sleep onset (17). “2011 Sleep in America” also reported that more than 90% individuals used digital screens within 1–h before sleep (17). Thus, the eyes are increasingly exposed to electronic screens or artificial light (17), which contributes to various diseases, including male infertility (Figure 2).
A retrospective study recently investigated the impact of digital screen on sperm quality in 116 men (17). Tablet or smartphone usage in the evening or after bedtime was associated with decreased total motile sperm number (−0.173, p < 0.05), sperm progressive motility (−0.322, −0.299, p < 0.05), sperm concentration (−0.169, p < 0.05), and motility (−0.392, −0.369, p < 0.05) while increasing the percentage of immotile sperm (0.382, 0.344, p < 0.05) (17). The authors hypothesized that artificial light chronically impaired the wake/sleep cycle and disturbed the circadian rhythm, which finally deteriorated male fertility by suppressing melatonin levels (17). However, the exact mechanism has not been explored and other artificial illuminations were not analyzed.
Ogo et al. divided pregnant rats into groups of constant light–light (LL) or light–dark (LD) cycles during the gestation period, but all offspring were housed under a normal LD photoperiod until adulthood (57). Offspring in the constant LL group showed a significantly increased number of abnormal sperm heads and decreased normal sperm number (p = 0.0001). The testosterone level, seminiferous tubule diameter, Sertoli cell number, and sperm count in the epididymis were also decreased (57). Moreover, LL reduced glutathione reductase (GR) levels in the epididymis but increased the enzymatic activity of glutathione S-transferase (GST) and glutathione peroxidase (GPx) in the testis (57). These findings suggest that extended light exposure leads to male infertility by decreasing testosterone levels and increasing oxidative stress (57). Moustafa et al. also placed male rats under prolonged light (20-h light and 4-h dark) and darkness (4-h light and 20-h dark) for 12 weeks (57). The prolonged light increased sperm count and motility, while extended darkness reduced the incidence of sperm abnormalities (57). Meanwhile, both extended light and darkness altered hormone secretion and reduced estradiol levels, while increasing FSH, LH, testosterone, and prolactin levels (57). Furthermore, prolonged light exposure increased the expression of Per1/2 and decreased Bmal1, while darkness exposure upregulated Per1/2, Clock, and Rer-erab (57). These results proved that abnormal light exposure disturbed testosterone secretion and the testicular circadian rhythm (57).
Similar to impaired female reproduction, abnormal light exposure may also lead to male infertility (17, 28, 57). However, current results on sperm parameters, hormone levels, and antioxidant indicators are diverse and controversial, while the specific mechanisms of how altered light exposure contributes to male infertility remain to be explored.
4 Shiftwork and male fertility
Sleep is a repetitive behavior that synchronizes with daily circadian rhythms, and a regular wake/sleep cycle is essential for human health (29). Shiftwork refers to working beyond the traditional daytime (08:00 to 16:00), which covers rotating or non-rotating (fixed) shift models (29, 58). The European Working Condition Survey in 2005 estimated that more than 17% of staff in the European Union performed shiftwork (29). Several studies have shown that shiftwork disturbed the wake/sleep cycle and impaired circadian rhythms, which contributed to cardiovascular disorders, metabolic diseases, and male infertility (29, 59) (Figure 2).
Demirkol et al. found that shift workers (n = 104) had a higher incidence of oligozoospermia (p = 0.006) and lower mean normal morphology (p = 0.036) than non-shift workers (n = 116), while shiftwork (OR = 2.11, 95% CI: 1.03 to 4.34) was independently related to oligozoospermia in multivariate analysis (29). Kohn et al. claimed that shift workers had lower sperm density (p = 0.012), total motile count (p = 0.019), and testosterone levels (p = 0.026) than non-shift workers, whereas no difference was observed in sperm volume and motility or FSH and LH levels (60). El-Helaly et al. explored the impact of occupational exposure on male fertility and found that shiftwork significantly increased the risk of infertility (OR = 3.60, 95% CI: 1.12 to 11.57) (61), which was consistent with findings of Irgens et al. who reported reduced sperm quality among shift workers (OR = 1.46, 95% CI: 0.89 to 2.40) (61). Sheiner et al. also revealed that male infertility was significantly associated with shiftwork (OR = 3.12, 95% CI: 1.19 to 8.13) (62). However, the mechanism by which shiftwork impairs male fertility requires further analysis.
Furthermore, a cross-sectional study with 1,346 men found that rotating shift workers (RSW) had significantly lower total sperm counts (median: 147.3 × 106, interquartile range (IQR): 80.7 × 106 to 255.3 × 106) than day workers (median: 176 × 106, IQR: 101.9 × 106 to 281.2 × 106) (p = 0.034) (63). More RSW (42.4%) revealed lower total sperm count (≤120 × 106) than day workers (30.5%) (p = 0.005); this remained true even after controlling for age, income, smoking, alcohol consumption, BMI, educational level, and abstinence period (OR = 1.60, 95% CI: 1.10 to 2.32, p = 0.014) (63). For permanent shift workers (PSW), LH was the only significantly different parameter (p = 0.044) when compared with day workers (63). In consideration of these differences between the two shift working types, the authors suggested that PSW might align their wake/sleep cycle on workdays with their schedule on days off, which finally decreased circadian desynchrony (63). However, this hypothesis remains unexplored. In addition, a study using a mouse model exposed to photoperiod shifting (light on at 20:00 and off at 08:00) for 28 days showed that the mean total sperm count was significantly decreased by inducing apoptosis in seminiferous tubules, while sperm parameters recovered when light shifting was altered to a constant normal photoperiod for another 35 days (63). However, whether such recovery of sperm quality could be observed in shift workers requires more evidence.
In contrast, Tuntiseranee et al. (64) and Bisanti et al. (65) reported that night shiftwork was not related to male infertility, while another study with 456 males also claimed that shiftwork or night work did not alter sperm quality (66). Considering this discrepancy, further studies with large prospective populations are necessary to verify the impact of shiftwork on male fertility before providing suggestions to shiftwork schedules.
5 Sleep duration and male fertility
Similar to proper diet and exercise, normal sleep duration is important for human health (67). The National Sleep Foundation has suggested a sleep period of 7–9 h for people aged 18–64 years and 7–8 h for those over 65 years (68, 69). However, on overloaded work schedule and environmental light/noise pollution, the National Health Interview Survey found that 30% workers (nearly 40.6 million) sleep for <6 h/night in the US (70). Longitudinal studies have claimed that insufficient sleep promoted the risks of mortality, hypertension, T2DM, obesity, and myocardial infraction (69), while others reported a U-shaped association that sleeping for 7–9 h/night had the least risk of deteriorating human health (69, 71). The effect of sleep duration on male fertility has also been explored (69) (Figure 2).
Shi et al. enrolled 328 males and found that the sperm concentration remained constant when sleeping for 4.7–8.0 h/night; however, it remarkably dropped when sleeping for <4.7 h/night and noticeably increased when sleeping for >8 h/night (31). Liu et al. observed that sperm count, motility, and survival rates were significantly decreased in participants sleeping for <6 h/night when compared with average (7–8 h/night) or long (>9 h/night) durations (5, 20). Demirkol et al. also reported a positive correlation between sleep duration and sperm concentration in 104 shift workers (29). These results suggest that insufficient sleep significantly impairs sperm quality.
In contrast, an inverse U-shaped relationship between sleep duration and male fertility was observed (72). The Pregnancy Online Study (PRESTO) with 1,176 couples revealed that the fecundability ratio (FRs) for 8 h/night was significantly higher than that for <6 h/night (FR: 0.62, 95% CI: 0.45–0.87), 7 h/night (FR: 0.97, 95% CI: 0.81–1.17), and ≥9 h/night (FR: 0.73, 95% CI: 0.46–1.15), indicating 8 h/night as the most suitable sleep duration for male fertility (73). The Male Reproductive Health in Chongqing College Students (MARHCS) study, which enrolled 796 individuals in 2013, showed that the highest sperm parameters were found for sleeping for 7.0–7.5 h/night, while both shorter and longer sleep durations were correlated with a declined sperm index (p = 0.001 and 0.002, respectively) in a dose-response manner (72). Sleeping for ≤6.5 h/night was related to a 4.6% (95% CI: -10.5 to 22.3) reduction in sperm volume and a 25.7% (95% CI: -1.2 to 60.1) reduction in total sperm number, while sleeping for >9.0 h/night was correlated with 21.5% (95% CI: 9.2 to 32.2) and 39.4% (95% CI: 23.3 to 52.1) reductions, respectively (72). Moreover, sperm parameters were significantly improved when sleep duration was altered to 7.0–7.5 h/night in the following year, which confirmed the sleep–fertility association again (72). However, this damage to sperm quality might not be completely reversible or need more time to recover, as Chen et al. revealed that improved sperm quality was only found in a small (but significant) proportion of patients who changed the sleep duration to a “proper” length (72). High DNA stainability (HDS) in the epididymis is an important index of incompletely differentiated sperms (74). Wang et al. reported that HDS was highest when sleeping for 7.0–7.5 h/night, while those with both shorter and longer sleep durations presented lower HDS (71). A preliminary cross-sectional study based on 92 healthy men also demonstrated an inverse U-shaped relationship between sleep duration and testicular volume, with those sleeping for 9.5 h/night showing the largest volume (75).
These epidemiological studies indicate that sleep duration is strongly associated with male fertility and sperm quality, although the specific positive, negative, or inverse U-shaped association remains to be clarified. Moreover, evidence of long sleep duration impairing male fertility is relatively weak and inaccurate.
From the perspective of animal research, sleep restriction (SR) to 6 h/night (sleeping from 10:00 to 16:00) for 21 days significantly increased immotile spermatozoa and impaired sperm motility, whereas total sperm number and transit time through the epididymis did not change (76). Chen et al. observed that SR for 35 days decreased sperm concentration, viability, and motility, while it increased sperm malformation (77). Alvarenga et al. found that SR and paradoxical sleep deprivation (PSD) resulted in 15% and 50% lower sperm viability, respectively; although sperm concentration was similar, spermatozoa with faster movement were significantly decreased compared to the control group (78). Moreover, Rizk et al. showed that PSD for 5 days significantly increased abnormal sperm morphology but decreased sperm count, viability, and motility (30). Choi et al. reported that SD for 7 days (SD7) significantly reduced sperm motility, whereas SD4 and SD7 partially induced seminiferous tubular atrophy and spermatid retention (32). These results suggest that sleep deficiency impairs sperm viability by disrupting sperm cycle maintenance (78); however, the specific mechanism remains to be investigated.
As sperm quality is mainly regulated by hormonal concentration, numerous studies have focused on the relationship between sleep duration and hormonal levels. The testosterone level is the highest in the morning and lowest during evening, and numerous clinical and basic investigations have shown that sleep deficiency/restriction altered the testosterone concentration by disturbing its secreted cycle (23, 69, 79–81). For example, Chen et al. found that sleeping for 6 h/night for 35 days significantly decreased the testosterone concentration (77) and Alvarenga et al. observed that SD for 96 h significantly inhibited testosterone levels by 45% (78). Choi et al. reported that SD4 and SD7 significantly decreased testosterone release but increased corticosterone production (32). Although the mechanism has not been clarified (77), authors inferred that sleep deficiency increased cortisol and corticosterone levels by activating the hypothalamic–pituitary–adrenal (HPA) axis, the feedback of which inhibited the hypothalamus–pituitary–gonadal (HPG) axis to decrease testosterone secretion (30, 32). In contrast, a preliminary cross-sectional study based on 92 healthy men found that insufficient sleep did not alter total/free testosterone levels (75), while the MARHCS trial found that sleep duration had no impact on reproductive hormones (72). Siervo et al. even found that sleep deficiency significantly increased the testosterone concentration in plasma and intratesticle (76, 82). Some authors thus thought that the altered hormones might not be responsible for decreased sperm quality or infertility (72). As sleep deficiency inhibits testosterone secretion during the second half of a biological night (69, 81), others have suggested that different types and definitions of sleep deficiency may contribute to the controversial interaction between sleep duration and male fertility (69).
Sleep deficiency also damages testes by inducing oxidative stress (76, 82). Akindele et al. found that SR for 14 days significantly elevated testicular malondialdehyde and glutathione (GSH) in adult rats (82, 83). Siervo et al. revealed that SR for 21 days sharply increased the tert-butyl hydroperoxide-initiated chemiluminescence (CL) curve of the epididymidis, which suggested an enhanced peroxidative attack by ROS (76, 82). These results infer that sleep deficiency may impair sperm quality by disrupting the balance between oxidative and antioxidant stress (30, 76). Another male Wistar rat model found that SD (sleeping for 4 h/night) impaired functions of the blood–testis and blood–epididymis barriers by increasing its permeability to low/high-molecular-weight tracers and decreasing the expression of tight-junction proteins, androgen, and actin receptors (84). In this model, rat fertility was improved by recovering sleep for 2–3 days, as the percentage of ejaculating males and impregnated females increased (84).
In general, although more studies tend to show that sleep duration plays an essential role in male fertility and sleep deficiency contributes to infertility, some authors still disagree on the aforementioned correlation as the research designs are diverse and the results are inconsistent. Moreover, exploring how sleep duration regulates male fertility is also a huge challenge considering the complex mechanisms of reproductive function. Finally, whether prolonged sleep duration restores sperm quality remains to be proved.
6 Sleep quality and male fertility
Growing evidence has suggested that sleep quality had a significant impact on human health, while poor sleep [defined as difficulties in falling asleep and lying awake at night (5, 85)] increased the risk of hypertension, T2DM, cardiovascular disease, depression, cancer, and male infertility (85) (Figure 2).
In 2013, Jensen et al. explored the association between sleep disturbance (based on the Karolinska Sleep Questionnaire) and semen quality in 953 young Danish men (16, 85). Males with both lower and higher sleep scores had significantly decreased total sperm count and concentration, percentage of motile spermatozoa and morphologically normal spermatozoa, and testis size than the control group (sleep score: 11 to 20) (16, 85). Those with a sleep score >50 (poor sleep) had a 29% reduction (95% CI: 2 to 48) in sperm concentration, 25% decline (95% CI: -4 to 46) in total sperm count, 0.9% lower (95% CI: -3.1 to 4.9) motile spermatozoa, and 1.6% fewer (95% CI: 0.3 to 3.0) morphologically normal spermatozoa compared to the control group (16, 85). Kohn et al. also found an inverse U-shaped relationship between sleep quality and sperm quality; the total motile count for moderate sleep quality was 15.4 M sperm/ml greater than individuals without sleep difficulty and 4.72 M sperm/ml greater than those with severe sleep difficulty (p = 0.018) (60).
Some authors have previously evaluated sleep quality using the Pittsburgh Sleep Quality Index (PSQI) global scores. For instance, Chen et al. recruited 842 healthy males which revealed that poor sleep quality (PSQI >5.0) had a lower total sperm count (8.0%, 95% CI: -15% to -0.046%), motility (3.9%, 95% CI: −6.2% to −1.5%), and progressive motility (4.0%, 95% CI: −6.5% to −1.4%) (86). Du et al. conducted a cross-sectional study among 970 patients and demonstrated that increased PSQI scores indicated lower total sperm number (r = −0.160008, p < 0.001), sperm concentration (r = −0.167063, p < 0.001), motility (r = −0.187979, p < 0.001), progressive motility (r = −0.192902, p < 0.001), and normal morphology (r = −0.124511, p < 0.001) (5). Hvidt et al. analyzed the fertility of 140 males and similarly found that sperm quality was reduced when PSQI was increased (p = 0.04) (85). Meanwhile, Viganò et al. observed that sperm motility was decreased for patients lying awake most of the night, whereas sperm volume was lower and concentration was higher for those with difficulty in initiating sleep (87).
Studies have suggested that poor sleep quality might decrease the serum testosterone concentration (86, 88) and impair Sertoli cells in the seminiferous tubules (32, 86). However, Jensen et al. (16), Chen et al. (72), Ruge et al. (89), and Du et al. (5) did not observe any correlation between sleep quality and reproductive hormone levels. In general, although some authors speculated that poor sleep quality (86, 90) or inappropriate sleep habits (5, 38, 91) disturbed the circadian rhythms and damaged male fertility, the results were still inconsistent and the evidence was weak. Further epidemiological and fundamental studies are required to clarify this issue.
7 Circadian/circannual rhythms and male fertility
As the two most prominent biological rhythms, day/night alterations (circadian rhythm) and seasonal changes (circannual rhythm) play essential roles in numerous biological functions, including male fertility or sperm quality (24) (Figure 2).
The MARHCS dataset, which analyzed 10,362 community populations, found that the sperm DNA fragmentation index (DFI, the most frequent parameter to assess sperm chromatin integrity) decreased from 08:00 to 11:00 (p = 0.335) and then increased after 12:00 (p < 0.001), while the DFI was elevated by 4.2% per hour (95% CI: 1.9 to 6.7, p < 0.001) after 11:00 (92). The Reproductive Medical Center (RMC) dataset with 630 clinical populations showed that sperm DFI decreased before 11:00 (p < 0.01) and each hour (after 07:00) was associated with a 3.8% reduction (92). The rat model proved that sperm DFI varied at different times (p = 0.05); it decreased from 03:00 to 07:00 (p = 0.038) and then increased until 23:00 (p = 0.002) (92). Further cosinor analysis indicated a nadir of DFI at 10:00 (92). Xie et al. analyzed 12,245 sperm samples and found that semen collected before 07:00 had the highest total sperm count and concentration, as well as normal sperm morphology (24). Moreover, Shimomura et al. observed that the total motile sperm count and total sperm count were significantly higher in samples collected in the evening than in the morning (34). This suggests that sperm quality was lower in the morning, while evening-collected semen might be easier to achieve successful intrauterine insemination (34).
The circannual rhythm also significantly influences reproductive behavior, especially in mammals (24). To guarantee the greatest survival chances, many species have adapted to ensure that offspring are born at the most suitable time of the year, when climate and food are the most favorable (24). Although humans are not seasonal breeders, sexual activity (24, 25) and reproductive function still alter with a circannual rhythm (24, 26). Xie et al. analyzed 12,245 semen samples and proved that total sperm count and concentration were higher in spring and lower in summer, while morphologically normal spermatozoids were significantly increased in summer (24). The authors suggested that photoperiod alteration was the most likely cause of circannual variation in sperm quality (24, 93), while temperature variation might be another reason for high hyperpyrexia impaired spermatogenesis (24, 93). Circadian clock genes were also involved, as Akiyama et al. reported that Bmal1 expression in the hypothalamus and testes was significantly decreased in the transitional season compared to the reproductive and non-reproductive seasons; the Cry1 level also sharply declined in the transitional season compared to the reproductive season. The testicular morphology and circadian clock genes (Bmal1 and Cry1) revealed circannual alterations (94). However, whether and how circadian clock genes regulate male fertility in circannual rhythms requires further exploration.
8 Body temperature and male fertility
Similar to light alteration and wake/sleep cycle, daily oscillation of environmental temperature is another typical Zeitgeber to synchronize the internal circadian system; thus, body temperature also presents a 24-h oscillation to adapt to the Earth’s rotation (95). High ambient temperatures can reduce sperm production by destroying the spermatogenic epithelial structure, inducing testicular oxidative stress, and promoting germ cell apoptosis (95–98). Meanwhile, hyperthermia regulates testicular function by altering the daily secretion of reproductive hormones and changing the expression of circadian and testosterone synthesis-related genes (95) (Figure 2).
Li et al. exposed male mice to hyperthermia (39°C) from 11:00 to 15:00 (4 h/day) for 35 days (95). This hyperthermia disturbed the rhythm of testosterone secretion, which increased at 11:00 h and decreased at 15:00 h (95). Hyperthermia promoted the transcription of testicular Star and Ar at 11:00 and enhanced the protein level of CYP11A1 at 23:00 (95). Moreover, heat exposure stimulated testicular Clock expression but decreased its protein content at 11:00 and increased the BMAL1 concentration at 23:00 (95). This suggests that high external temperature arrests spermatogenesis by disrupting the rhythms of testosterone secretion and clock genes (95). Sabés-Alsina et al. maintained New Zealand White rabbits under environmental temperatures increasing from 22°C to 31°C (maintained for 3 h) and then gradually declined to 22°C until 09:00 on the following day (99). Hyperthermia significantly decreased the percentage of viable spermatozoa (74.21% vs. 80.71%) (p < 0.05) and increased the ratio of acrosomic abnormalities (36.96% vs. 22.57%) and tailless spermatozoa (12.83% vs. 7.91%) (p < 0.01) (99). However, heat exposure did not alter motility parameters or fertility and prolificacy rates (99). The lack of impairment on fertility and prolificacy may be due to the rapid recovery of reproductive function (99).
In summary, studies on the impact of environmental temperature on sperm quality are diverse and definite; however, whether the oscillation of body temperature influences male fertility and, if so, how it works remains a mystery.
9 Clock genes and testosterone synthesis
Although the diverse reproductive hormones are involved in regulating male fertility and facilitating the spermatogenetic process, only testosterone is essential to maintaining spermatogenesis (100). Mainly released by Leydig cells, the serum testosterone concentration presents rhythmic oscillation in adult male mice and human (101–104) which starts to rise at sleep onset (peaks around 8:00) but falls during the day (trough around 20:00) (104, 105). It is reported that circadian misalignment shifts the summit value of the testosterone’s diurnal rhythm to happen soon after waking up (106); however, whether circadian disturbance alters the testosterone concentration is controversial (5, 15, 85, 104, 106–108) and which clock element regulates its secretion is also unknown (103) (Figure 2).
Alvarez et al. have found that BMAL1 protein was rhythmically expressed in mouse Leydig cells (103). Since then, most of the core clock genes like Bmal1, Per1/2/3, Cry1/2, Rev-erbα/β, Rorb, and Dbp in Leydig cells were demonstrated to rhythmically oscillate (101–103, 109), except Clock, Rora, Ck1δ, and Ck1ε (102). Meanwhile, the steroidogenic-related genes which are responsible for testosterone production in Leydig cells (including Star, Cyp11a1, Cyp17a1, Hsd3b2, Hsd17b3, Sf1, positive-Nur77, and negative-Arr19) also exhibited 24-h rhythmic expression patterns (101, 102, 109, 110). Specifically, Baburski et al. found the summits of Star, Cyp11a1, and Cyp17a1 oscillation occurred approximately at the middle of the light phase, i.e., a few hours before the testosterone release peak (102). These results indicate a crucial role of the circadian clock in testosterone production (101–103, 110). Furthermore, Bmal1 knockout or inhibition was reported to decrease testosterone secretion by reducing mRNA levels of steroidogenic genes (Star, Cyp11a1, Hsd3b2, Hsd17b3, 3β-Hsd, Sf1, and Nur77) (101, 103, 111, 112) and Apo (Apoa1/2 and Apoc3) (112). Moreover, Bmal1 knockdown inhibited testosterone level by inducing apoptosis of Leydig cells (111), and the circadian clock system was involved to the process of bisphenol A (113) and zearalenone (114) reducing testosterone production. In a word, circadian rhythms can regulate testosterone production by various signaling pathways.
10 Conclusion
The circadian rhythm is strongly correlated with human health, while growing evidence suggests that circadian disorders contribute to male infertility. With the high incidence of night shiftwork, sleep deficiency, and poor sleep quality in modern life, numerous studies have investigated their influences on fertility and found that they impaired sperm quality and increased the risk of male infertility. Evidence for the impacts from light, body temperature, and circadian/circannual rhythms is relatively weak, although some correlations have been uncovered. However, the current conclusions were inconsistent as the abundant indices of sperm quality and male reproduction, while how the circadian clock genes were involved also remained to be further explored. Nevertheless, a better understanding on the interaction between circadian rhythm disturbance and male fertility will be meaningful, as a more scientific and rational lifestyle and work schedule might help to improve infertility.
Author contributions
All authors researched data, made substantial contributions to discussion content, and edited the manuscript before submission. TL, YB and YJ wrote the article, JG and FS guided and revised it. All authors contributed to the article and approved the submitted version.
Funding
This manuscript was funded by the National Nature Science Foundation of China (No. 82060276) and the Science and Technology Department of Guizhou Province [QianKeHeJiChu-ZK(20210)YiBan382].
Conflict of interest
The authors declare that the research was conducted in the absence of any commercial or financial relationships that could be construed as a potential conflict of interest.
Publisher’s note
All claims expressed in this article are solely those of the authors and do not necessarily represent those of their affiliated organizations, or those of the publisher, the editors and the reviewers. Any product that may be evaluated in this article, or claim that may be made by its manufacturer, is not guaranteed or endorsed by the publisher.
References
1. Noh JY, Han DH, Yoon JA, Kim MH, Kim SE, Ko IG, et al. Circadian rhythms in urinary functions: possible roles of circadian clocks? Int Neurourol J (2011) 15(2):64–73. doi: 10.5213/inj.2011.15.2.64
2. Chang WH, Lai AG. Timing gone awry: distinct tumour suppressive and oncogenic roles of the circadian clock and crosstalk with hypoxia signalling in diverse malignancies. J Transl Med (2019) 17(1):132. doi: 10.1186/s12967-019-1880-9
3. Walker W, Bumgarner J, Walton J, Liu J, Meléndez-Fernández O, Nelson R, et al. Light pollution and cancer. Int J Mol Sci (2020) 21(24):9360. doi: 10.3390/ijms21249360
4. Shostak A. Circadian clock, cell division, and cancer: From molecules to organism. Int J Mol Sci (2017) 18(4):873. doi: 10.3390/ijms18040873
5. Du CQ, Yang YY, Chen J, Feng L, Lin WQ. Association between sleep quality and semen parameters and reproductive hormones: A cross-sectional study in zhejiang, China. Nat Sci Sleep (2020) 12:11–8. doi: 10.2147/nss.s235136
6. Manoogian ENC, Panda S. Circadian rhythms, time-restricted feeding, and healthy aging. Ageing Res Rev (2017) 39:59–67. doi: 10.1016/j.arr.2016.12.006
7. Xu H, Huang L, Zhao J, Chen S, Liu J, Li G. The circadian clock and inflammation: A new insight. Clin Chim Acta (2020) 512:12–7. doi: 10.1016/j.cca.2020.11.011
8. Panda S. Circadian physiology of metabolism. Science (2016) 354(6315):1008–15. doi: 10.1126/science.aah4967
9. Chan MC, Spieth PM, Quinn K, Parotto M, Zhang H, Slutsky AS. Circadian rhythms: from basic mechanisms to the intensive care unit. Crit Care Med (2012) 40(1):246–53. doi: 10.1097/CCM.0b013e31822f0abe
10. Ulgherait M, Midoun AM, Park SJ, Gatto JA, Tener SJ, Siewert J, et al. Circadian autophagy drives iTRF-mediated longevity. Nature (2021) 598(7880):353–8. doi: 10.1038/s41586-021-03934-0
11. McAlpine CS, Swirski FK. Circadian influence on metabolism and inflammation in atherosclerosis. Circ Res (2016) 119(1):131–41. doi: 10.1161/circresaha.116.308034
12. Garcia-Saenz A, Sánchez de Miguel A, Espinosa A, Valentin A, Aragonés N, Llorca J, et al. Evaluating the association between artificial light-at-Night exposure and breast and prostate cancer risk in Spain (MCC-Spain study). Environ Health Perspect (2018) 126(4):047011. doi: 10.1289/EHP1837
13. Tobaldini E, Costantino G, Solbiati M, Cogliati C, Kara T, Nobili L, et al. Sleep, sleep deprivation, autonomic nervous system and cardiovascular diseases. Neurosci Biobehav Rev (2017) 74(Pt B):321–9. doi: 10.1016/j.neubiorev.2016.07.004
14. Masri S, Sassone-Corsi P. The emerging link between cancer, metabolism, and circadian rhythms. Nat Med (2018) 24(12):1795–803. doi: 10.1038/s41591-018-0271-8
15. Vignozzi L, Maggi M. Circadian rhythm and erectile function: is there a penile clock? Nat Rev Urol (2020) 17(11):603–4. doi: 10.1038/s41585-020-00376-7
16. Jensen TK, Andersson AM, Skakkebæk NE, Joensen UN, Blomberg Jensen M, Lassen TH, et al. Association of sleep disturbances with reduced semen quality: a cross-sectional study among 953 healthy young Danish men. Am J Epidemiol (2013) 177(10):1027–37. doi: 10.1093/aje/kws420
17. Green A, Barak S, Shine L, Kahane A, Dagan Y. Exposure by males to light emitted from media devices at night is linked with decline of sperm quality and correlated with sleep quality measures. Chronobiol Int (2020) 37(3):414–24. doi: 10.1080/07420528.2020.1727918
18. Buysse DJ, Reynolds CF 3rd, Monk TH, Berman SR, Kupfer DJ. The Pittsburgh sleep quality index: a new instrument for psychiatric practice and research. Psychiatry Res (1989) 28(2):193–213. doi: 10.1016/0165-1781(89)90047-4
19. Nordin M, Åkerstedt T, Nordin S. Psychometric evaluation and normative data for the karolinska sleep questionnaire. Sleep Biol Rhythms (2013) 11(4):216–26. doi: 10.1111/sbr.12024
20. Liu MM, Liu L, Chen L, Yin XJ, Liu H, Zhang YH, et al. Sleep deprivation and late bedtime impair sperm health through increasing antisperm antibody production: A prospective study of 981 healthy men. Med Sci Monit (2017) 23:1842–8. doi: 10.12659/msm.900101
21. Harrison Y, Horne JA. Long-term extension to sleep–are we really chronically sleep deprived? Psychophysiology (1996) 33(1):22–30. doi: 10.1111/j.1469-8986.1996.tb02105.x
22. Roehrs T, Shore E, Papineau K, Rosenthal L, Roth T. A two-week sleep extension in sleepy normals. Sleep (1996) 19(7):576–82. doi: 10.1093/sleep/19.7.576
23. Lateef OM, Akintubosun MO. Sleep and reproductive health. J Circadian Rhythms (2020) 18:1. doi: 10.5334/jcr.190
24. Xie M, Utzinger KS, Blickenstorfer K, Leeners B. Diurnal and seasonal changes in semen quality of men in subfertile partnerships. Chronobiol Int (2018) 35(10):1375–84. doi: 10.1080/07420528.2018.1483942
25. Saint Pol P, Beuscart R, Leroy-Martin B, Hermand E, Jablonski W. Circannual rhythms of sperm parameters of fertile men. Fertil Steril (1989) 51(6):1030–3. doi: 10.1016/s0015-0282(16)60738-0
26. Roenneberg T, Aschoff J. Annual rhythm of human reproduction: I. Biology sociology both? J Biol Rhythms (1990) 5(3):195–216. doi: 10.1177/074873049000500303
27. Caetano G, Bozinovic I, Dupont C, Léger D, Lévy R, Sermondade N. Impact of sleep on female and male reproductive functions: a systematic review. Fertil Steril (2021) 115(3):715–31. doi: 10.1016/j.fertnstert.2020.08.1429
28. Moustafa A. Effect of light-dark cycle misalignment on the hypothalamic-Pituitary-Gonadal axis, testicular oxidative stress, and expression of clock genes in adult Male rats. Int J Endocrinol (2020) 2020:1426846. doi: 10.1155/2020/1426846
29. Demirkol MK, Yıldırım A, Gıca Ş., Doğan NT, Resim S. Evaluation of the effect of shift working and sleep quality on semen parameters in men attending infertility clinic. Andrologia (2021) 53(8):e14116. doi: 10.1111/and.14116
30. Rizk NI, Rizk MS, Mohamed AS, Naguib YM. Attenuation of sleep deprivation dependent deterioration in male fertility parameters by vitamin c. Reprod Biol Endocrinol (2020) 18(1):2. doi: 10.1186/s12958-020-0563-y
31. Shi X, Chan CPS, Waters T, Chi L, Chan DYL, Li TC. Lifestyle and demographic factors associated with human semen quality and sperm function. Syst Biol Reprod Med (2018) 64(5):358–67. doi: 10.1080/19396368.2018.1491074
32. Choi J, Lee S, Bae J, Shim J, Park H, Kim Y, et al. Effect of sleep deprivation on the Male reproductive system in rats. J Korean Med Sci (2016) 31(10):1624–30. doi: 10.3346/jkms.2016.31.10.1624
33. Agarwal A, Mulgund A, Hamada A, Chyatte MR. A unique view on male infertility around the globe. Reprod Biol Endocrinol (2015) 13:37. doi: 10.1186/s12958-015-0032-1
34. Shimomura Y, Shin T, Osaka A, Inoue Y, Iwahata T, Kobori Y, et al. Comparison between semen parameters in specimens collected early in the morning and in the evening. Syst Biol Reprod Med (2020) 66(2):147–50. doi: 10.1080/19396368.2020.1727994
35. Konopka RJ, Benzer S. Clock mutants of drosophila melanogaster. Proc Natl Acad Sci U.S.A. (1971) 68(9):2112–6. doi: 10.1073/pnas.68.9.2112
36. Dibner C, Schibler U, Albrecht U. The mammalian circadian timing system: Organization and coordination of central and peripheral clocks. Annu Rev Physiol (2010) 72:517–49. doi: 10.1146/annurev-physiol-021909-135821
37. Golombek DA, Rosenstein RE. Physiology of circadian entrainment. Physiol Rev (2010) 90(3):1063–102. doi: 10.1152/physrev.00009.2009
38. Gamble K, Resuehr D, Johnson C. Shift work and circadian dysregulation of reproduction. Front Endocrinol (Lausanne) (2013) 4:92. doi: 10.3389/fendo.2013.00092
39. Kriegsfeld LJ, LeSauter J, Silver R. Targeted microlesions reveal novel organization of the hamster suprachiasmatic nucleus. J Neurosci (2004) 24(10):2449–57. doi: 10.1523/jneurosci.5323-03.2004
40. van den Pol AN, Tsujimoto KL. Neurotransmitters of the hypothalamic suprachiasmatic nucleus: immunocytochemical analysis of 25 neuronal antigens. Neuroscience (1985) 15(4):1049–86. doi: 10.1016/0306-4522(85)90254-4
41. Mohawk JA, Green CB, Takahashi JS. Central and peripheral circadian clocks in mammals. Annu Rev Neurosci (2012) 35:445–62. doi: 10.1146/annurev-neuro-060909-153128
42. Giebultowicz J. Chronobiology: biological timekeeping. Integr Comp Biol (2004) 44(3):266. doi: 10.1093/icb/44.3.266
43. Abrahamson EE, Moore RY. Suprachiasmatic nucleus in the mouse: retinal innervation, intrinsic organization and efferent projections. Brain Res (2001) 916(1-2):172–91. doi: 10.1016/s0006-8993(01)02890-6
44. Deurveilher S, Burns J, Semba K. Indirect projections from the suprachiasmatic nucleus to the ventrolateral preoptic nucleus: a dual tract-tracing study in rat. Eur J Neurosci (2002) 16(7):1195–213. doi: 10.1046/j.1460-9568.2002.02196.x
45. Deurveilher S, Semba K. Indirect projections from the suprachiasmatic nucleus to major arousal-promoting cell groups in rat: Implications for the circadian control of behavioural state. Neuroscience (2005) 130(1):165–83. doi: 10.1016/j.neuroscience.2004.08.030
46. Schwartz MD, Urbanski HF, Nunez AA, Smale L. Projections of the suprachiasmatic nucleus and ventral subparaventricular zone in the Nile grass rat (Arvicanthis niloticus). Brain Res (2011) 1367:146–61. doi: 10.1016/j.brainres.2010.10.058
47. Challet E. Minireview: Entrainment of the suprachiasmatic clockwork in diurnal and nocturnal mammals. Endocrinology (2007) 148(12):5648–55. doi: 10.1210/en.2007-0804
48. Hastings MH, Maywood ES, Reddy AB. Two decades of circadian time. J Neuroendocrinol (2008) 20(6):812–9. doi: 10.1111/j.1365-2826.2008.01715.x
49. Zhang WX, Chen SY, Liu C. Regulation of reproduction by the circadian rhythms. Sheng Li Xue Bao (2016) 68(6):799–808. doi: 10.1080/07420528.2020.1727918
50. Hergenhan S, Holtkamp S, Scheiermann C. Molecular interactions between components of the circadian clock and the immune system. J Mol Biol (2020) 432(12):3700–13. doi: 10.1016/j.jmb.2019.12.044
51. Kloner RA, Speakman M. Erectile dysfunction and atherosclerosis. Curr Atheroscler Rep (2002) 4(5):397–401. doi: 10.1007/s11883-002-0078-3
52. Pilorz V, Astiz M, Heinen K, Rawashdeh O, Oster H. The concept of coupling in the mammalian circadian clock network. J Mol Biol (2020) 432(12):3618–38. doi: 10.1016/j.jmb.2019.12.037
53. Takeda N, Maemura K. The role of clock genes and circadian rhythm in the development of cardiovascular diseases. Cell Mol Life Sci (2015) 72(17):3225–34. doi: 10.1007/s00018-015-1923-1
54. Yamajuku D, Shibata Y, Kitazawa M, Katakura T, Urata H, Kojima T, et al. Cellular DBP and E4BP4 proteins are critical for determining the period length of the circadian oscillator. FEBS Lett (2011) 585(14):2217–22. doi: 10.1016/j.febslet.2011.05.038
55. Honma S, Kawamoto T, Takagi Y, Fujimoto K, Sato F, Noshiro M, et al. Dec1 and Dec2 are regulators of the mammalian molecular clock. Nature (2002) 419(6909):841–4. doi: 10.1038/nature01123
56. Anna G, Kannan NN. Post-transcriptional modulators and mediators of the circadian clock. Chronobiol Int (2021) 38(9):1244–61. doi: 10.1080/07420528.2021.1928159
57. Ogo FM, Siervo G, de Moraes AMP, Machado KGB, Scarton S, Guimarães ATB, et al. Extended light period in the maternal circadian cycle impairs the reproductive system of the rat male offspring. J Dev Orig Health Dis (2021) 12(4):595–602. doi: 10.1017/s2040174420000975
58. Presser HB. Job, family, and gender: determinants of nonstandard work schedules among employed americans in 1991. Demography (1995) 32(4):577–98. doi: 10.2307/2061676
59. Torquati L, Mielke GI, Brown WJ, Kolbe-Alexander T. Shift work and the risk of cardiovascular disease.A systematic review and meta-analysis including dose-response relationship. Scand J Work Environ Health (2018) 44(3):229–38. doi: 10.5271/sjweh.3700
60. Kohn T, Pastuszak A, Pickett S, Kohn J, Lipshultz L. PD13-08 shift work is associated with altered semen parameters in infertile men. J Urol (2017) 197:e273–4. doi: 10.1016/j.juro.2017.02.696
61. El-Helaly M, Awadalla N, Mansour M, El-Biomy Y. Workplace exposures and male infertility - a case-control study. Int J Occup Med Environ Health (2010) 23(4):331–8. doi: 10.2478/v10001-010-0039-y
62. Sheiner EK, Sheiner E, Carel R, Potashnik G, Shoham-Vardi I. Potential association between male infertility and occupational psychological stress. J Occup Environ Med (2002) 44(12):1093–9. doi: 10.1097/00043764-200212000-00001
63. Liu K, Hou G, Wang X, Chen H, Shi F, Liu C, et al. Adverse effects of circadian desynchrony on the male reproductive system: an epidemiological and experimental study. Hum Reprod (2020) 35(7):1515–28. doi: 10.1093/humrep/deaa101
64. Tuntiseranee P, Olsen J, Geater A, Kor-anantakul O. Are long working hours and shiftwork risk factors for subfecundity? a study among couples from southern Thailand. Occup Environ Med (1998) 55(2):99–105. doi: 10.1136/oem.55.2.99
65. Bisanti L, Olsen J, Basso O, Thonneau P, Karmaus W. Shift work and subfecundity: a European multicenter study. European study group on infertility and subfecundity. J Occup Environ Med (1996) 38(4):352–8. doi: 10.1097/00043764-199604000-00012
66. Eisenberg ML, Chen Z, Ye A, Buck Louis GM. Relationship between physical occupational exposures and health on semen quality: data from the longitudinal investigation of fertility and the environment (LIFE) study. Fertil Steril (2015) 103(5):1271–7. doi: 10.1016/j.fertnstert.2015.02.010
67. O'Byrne NA, Yuen F, Niaz W, Liu PY. Sleep and the testis. Curr Opin Endocr Metab Res (2021) 18:83–93. doi: 10.1016/j.coemr.2021.03.002
68. Hirshkowitz M, Whiton K, Albert SM, Alessi C, Bruni O, DonCarlos L, et al. National sleep foundation's sleep time duration recommendations: methodology and results summary. Sleep Health (2015) 1(1):40–3. doi: 10.1016/j.sleh.2014.12.010
69. Liu PY. A clinical perspective of sleep and andrological health: Assessment, treatment considerations, and future research. J Clin Endocrinol Metab (2019) 104(10):4398–417. doi: 10.1210/jc.2019-00683
70. Weingarten JA, Collop NA. Air travel: effects of sleep deprivation and jet lag. Chest (2013) 144(4):1394–401. doi: 10.1378/chest.12-2963
71. Wang X, Chen Q, Zou P, Liu T, Mo M, Yang H, et al. Sleep duration is associated with sperm chromatin integrity among young men in chongqing, China. J Sleep Res (2018) 27(4):e12615. doi: 10.1111/jsr.12615
72. Chen Q, Yang H, Zhou N, Sun L, Bao H, Tan L, et al. Inverse U-shaped association between sleep duration and semen quality: Longitudinal observational study (MARHCS) in chongqing, China. Sleep (2016) 39(1):79–86. doi: 10.5665/sleep.5322
73. Wise LA, Rothman KJ, Wesselink AK, Mikkelsen EM, Sorensen HT, McKinnon CJ, et al. Male Sleep duration and fecundability in a north American preconception cohort study. Reprod Biol Endocrinol (2018) 109(3):453–9. doi: 10.1016/j.fertnstert.2017.11.037
74. Li M-W, Lloyd KCK. DNA Fragmentation index (DFI) as a measure of sperm quality and fertility in mice. Sci Rep (2020) 10(1):3833. doi: 10.1038/s41598-020-60876-9
75. Zhang W, Piotrowska K, Chavoshan B, Wallace J, Liu PY. Sleep duration is associated with testis size in healthy young men. J Clin Sleep Med (2018) 14(10):1757–64. doi: 10.5664/jcsm.7390
76. Siervo G, Ogo FM, Valério AD, Silva TNX, Staurengo-Ferrari L, Alvarenga TA, et al. Sleep restriction in wistar rats impairs epididymal postnatal development and sperm motility in association with oxidative stress. Reprod Fertil Dev (2017) 29(9):1813–20. doi: 10.1071/rd15535
77. Chen W, Guo X, Jin Z, Li R, Shen L, Li W, et al. Transcriptional alterations of genes related to fertility decline in male rats induced by chronic sleep restriction. Syst Biol Reprod Med (2020) 66(2):99–111. doi: 10.1080/19396368.2019.1678694
78. Alvarenga TA, Hirotsu C, Mazaro-Costa R, Tufik S, Andersen ML. Impairment of male reproductive function after sleep deprivation. Fertil Steril (2015) 103(5):1355–62.e1. doi: 10.1016/j.fertnstert.2015.02.002
79. Leproult R, Van Cauter E. Effect of 1 week of sleep restriction on testosterone levels in young healthy men. Jama (2011) 305(21):2173–4. doi: 10.1001/jama.2011.710
80. Reynolds AC, Dorrian J, Liu PY, Van Dongen HP, Wittert GA, Harmer LJ, et al. Impact of five nights of sleep restriction on glucose metabolism, leptin and testosterone in young adult men. PloS One (2012) 7(7):e41218. doi: 10.1371/journal.pone.0041218
81. Schmid SM, Hallschmid M, Jauch-Chara K, Lehnert H, Schultes B. Sleep timing may modulate the effect of sleep loss on testosterone. Clin Endocrinol (Oxf) (2012) 77(5):749–54. doi: 10.1111/j.1365-2265.2012.04419.x
82. Siervo G, Ogo FM, Staurengo-Ferrari L, Anselmo-Franci JA, Cunha FQ, Cecchini R, et al. Sleep restriction during peripuberty unbalances sexual hormones and testicular cytokines in rats. Biol Reprod (2019) 100(1):112–22. doi: 10.1093/biolre/ioy161
83. Akindele OO, Kunle-Alabi OT, Adeyemi DH, Oghenetega BO, Raji Y. Effects of vitamin e and melatonin on serum testosterone level in sleep deprived wistar rats. Afr J Med Med Sci (2014) 43(4):295–304.
84. Domínguez-Salazar E, Hurtado-Alvarado G, Medina-Flores F, Dorantes J, González-Flores O, Contis-Montes de Oca A, et al. Chronic sleep loss disrupts blood-testis and blood-epididymis barriers, and reduces male fertility. J Sleep Res (2020) 29(3):e12907. doi: 10.1111/jsr.12907
85. Hvidt J, Knudsen U, Zachariae R, Ingerslev H, Philipsen M, Frederiksen Y. Associations of bedtime, sleep duration, and sleep quality with semen quality in males seeking fertility treatment: a preliminary study. Basic Clin Androl (2020) 30:5. doi: 10.1186/s12610-020-00103-7
86. Chen H-G, Sun B, Chen Y-J, Chavarro JE, Hu S-H, Xiong C-L, et al. Sleep duration and quality in relation to semen quality in healthy men screened as potential sperm donors. Environ Int (2020) 135:105368. doi: 10.1016/j.envint.2019.105368
87. Viganò P, Chiaffarino F, Bonzi V, Salonia A, Ricci E, Papaleo E, et al. Sleep disturbances and semen quality in an Italian cross sectional study. Basic Clin Androl (2017) 27:16. doi: 10.1186/s12610-017-0060-0
88. Monder C, Sakai RR, Miroff Y, Blanchard DC, Blanchard RJ. Reciprocal changes in plasma corticosterone and testosterone in stressed male rats maintained in a visible burrow system: evidence for a mediating role of testicular 11 beta-hydroxysteroid dehydrogenase. Endocrinology (1994) 134(3):1193–8. doi: 10.1210/endo.134.3.8119159
89. Ohayon M, Carskadon M, Guilleminault C, Vitiello M. Meta-analysis of quantitative sleep parameters from childhood to old age in healthy individuals: developing normative sleep values across the human lifespan. Sleep (2004) 27(7):1255–73. doi: 10.1093/sleep/27.7.1255
90. Luboshitzky R, Zabari Z, Shen-Orr Z, Herer P, Lavie P. Disruption of the nocturnal testosterone rhythm by sleep fragmentation in normal men. J Clin Endocrinol Metab (2001) 86(3):1134–9. doi: 10.1210/jcem.86.3.7296
91. Boden M, Varcoe T, Kennaway D. Circadian regulation of reproduction: from gamete to offspring. Prog Biophys Mol Biol (2013) 113(3):387–97. doi: 10.1016/j.pbiomolbio.2013.01.003
92. Ni W, Liu K, Hou G, Pan C, Wu S, Zheng J, et al. Diurnal variation in sperm DNA fragmentation: analysis of 11,382 semen samples from two populations and in vivo animal experiments. Chronobiol Int (2019) 36(11):1455–63. doi: 10.1080/07420528.2019.1649275
93. Levine RJ, Brown MH, Bell M, Shue F, Greenberg GN, Bordson BL. Air-conditioned environments do not prevent deterioration of human semen quality during the summer. Fertil Steril (1992) 57(5):1075–83. doi: 10.1016/s0015-0282(16)55027-4
94. Akiyama M, Takino S, Sugano Y, Yamada T, Nakata A, Miura T, et al. Effect of seasonal changes on testicular morphology and the expression of circadian clock genes in japanese wood mice (Apodemus speciosus). J Biol Regul Homeost Agents (2015) 29(3):589–600.
95. Li Z, Li Y, Ren Y, Li C. High ambient temperature disrupted the circadian rhythm of reproductive hormones and changed the testicular expression of steroidogenesis genes and clock genes in male mice. Mol Cell Endocrinol (2020) 500:110639. doi: 10.1016/j.mce.2019.110639
96. Cameron RD, Blackshaw AW. The effect of elevated ambient temperature on spermatogenesis in the boar. J Reprod Fertil (1980) 59(1):173–9. doi: 10.1530/jrf.0.0590173
97. Li Y, Huang Y, Piao Y, Nagaoka K, Watanabe G, Taya K, et al. Protective effects of nuclear factor erythroid 2-related factor 2 on whole body heat stress-induced oxidative damage in the mouse testis. Reprod Biol Endocrinol (2013) 11:23. doi: 10.1186/1477-7827-11-23
98. Zhang M, Jiang M, Bi Y, Zhu H, Zhou Z, Sha J. Autophagy and apoptosis act as partners to induce germ cell death after heat stress in mice. PloS One (2012) 7(7):e41412. doi: 10.1371/journal.pone.0041412
99. Sabés-Alsina M, Planell N, Torres-Mejia E, Taberner E, Maya-Soriano M, Tusell L, et al. Daily exposure to summer circadian cycles affects spermatogenesis, but not fertility in an in vivo rabbit model. Theriogenology (2015) 83(2):246–52. doi: 10.1016/j.theriogenology.2014.09.013
100. Smith L, Walker W. The regulation of spermatogenesis by androgens. Semin Cell Dev Biol (2014) 30:2–13. doi: 10.1016/j.semcdb.2014.02.012
101. Xiao Y, Zhao L, Li W, Wang X, Ma T, Yang L, et al. Circadian clock gene BMAL1 controls testosterone production by regulating steroidogenesis-related gene transcription in goat leydig cells. J Cell Physiol (2021) 236(9):6706–25. doi: 10.1002/jcp.30334
102. Baburski A, Sokanovic S, Bjelic M, Radovic S, Andric S, Kostic T. Circadian rhythm of the leydig cells endocrine function is attenuated during aging. Exp Gerontol (2016) 73:5–13. doi: 10.1016/j.exger.2015.11.002
103. Alvarez J, Hansen A, Ord T, Bebas P, Chappell P, Giebultowicz J, et al. The circadian clock protein BMAL1 is necessary for fertility and proper testosterone production in mice. J Biol Rhythms (2008) 23(1):26–36. doi: 10.1177/0748730407311254
104. Cho JW, Duffy JF. Sleep, sleep disorders, and sexual dysfunction. World J Mens Health (2019) 37(3):261–75. doi: 10.5534/wjmh.180045
105. Andersen ML TS. The effects of testosterone on sleep and sleep-disordered breathing in men: its bidirectional interaction with erectile function. Sleep Med Rev (2008) 12(5):365–79. doi: 10.1016/j.smrv.2007.12.003
106. Liu PY. A clinical perspective of sleep and andrological health: Assessment, treatment considerations and future research. J Clin Endocrinol Metab (2019) 104(10):4398–417. doi: 10.1210/jc.2019-00683
107. Pastuszak AW, Moon YM, Scovell J, Badal J, Lamb DJ, Link RE, et al. Poor sleep quality predicts hypogonadal symptoms and sexual dysfunction in Male nonstandard shift workers. Urology (2017) 102:121–5. doi: 10.1016/j.urology.2016.11.033
108. Kirby W, Balasubramanian A, Santiago J, Hockenberry M, Skutt D, Kohn T, et al. MP91-06 increased risk of HYPOGONADAL symptoms in shift workers with shift work sleep disorder. J Urol (2017) 197:e1220. doi: 10.1016/j.juro.2017.02.2846
109. Baburski A, Sokanovic S, Janjic M, Stojkov-Mimic N, Bjelic M, Andric S, et al. Melatonin replacement restores the circadian behavior in adult rat leydig cells after pinealectomy. Mol Cell Endocrinol (2015) 413:26–35. doi: 10.1016/j.mce.2015.05.039
110. Chen H, Gao L, Xiong Y, Yang D, Li C, Wang A, et al. Circadian clock and steroidogenic-related gene expression profiles in mouse leydig cells following dexamethasone stimulation. Biochem Biophys Res Commun (2017) 483(1):294–300. doi: 10.1016/j.bbrc.2016.12.149
111. Ding H, Zhao J, Liu H, Wang J, Lu W. BMAL1 knockdown promoted apoptosis and reduced testosterone secretion in TM3 leydig cell line. Gene (2020) 747:144672. doi: 10.1016/j.gene.2020.144672
112. Yang L, Ma T, Zhao L, Jiang H, Zhang J, Liu D, et al. Circadian regulation of apolipoprotein gene expression affects testosterone production in mouse testis. Theriogenology (2021) 174:9–19. doi: 10.1016/j.theriogenology.2021.06.023
113. Li C, Zhang L, Ma T, Gao L, Yang L, Wu M, et al. Bisphenol a attenuates testosterone production in leydig cells via the inhibition of NR1D1 signaling. Chemosphere (2021) 263:128020. doi: 10.1016/j.chemosphere.2020.128020
Keywords: circadian rhythm, disturbance, male fertility, infertility, sperm quality
Citation: Li T, Bai Y, Jiang Y, Jiang K, Tian Y, Gu J and Sun F (2022) The potential impacts of circadian rhythm disturbances on male fertility. Front. Endocrinol. 13:1001316. doi: 10.3389/fendo.2022.1001316
Received: 23 July 2022; Accepted: 20 September 2022;
Published: 06 October 2022.
Edited by:
Qing Chen, Army Medical University, ChinaReviewed by:
Lucia Rocco, University of Campania Luigi Vanvitelli, ItalyHuatao Chen, Northwest A&F University, China
Copyright © 2022 Li, Bai, Jiang, Jiang, Tian, Gu and Sun. This is an open-access article distributed under the terms of the Creative Commons Attribution License (CC BY). The use, distribution or reproduction in other forums is permitted, provided the original author(s) and the copyright owner(s) are credited and that the original publication in this journal is cited, in accordance with accepted academic practice. No use, distribution or reproduction is permitted which does not comply with these terms.
*Correspondence: Fa Sun, sfgmc@sina.com; Jiang Gu, 570117957@qq.com
†These authors have contributed equally to this work